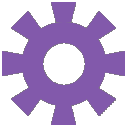
Browsing Pathways
Showing 64951 -
64960 of 605359 pathways
SMPDB ID | Pathway Name and Description | Pathway Class | Chemical Compounds | Proteins |
---|---|---|---|---|
SMP0000067 |
Aspartate MetabolismAspartate is synthesized by transamination of oxaloacetate by aspartate aminotransferase or amino acid oxidase. Aspartyl-tRNA synthetase can then couple aspartate to aspartyl tRNA for protein synthesis. The aspartate content in human proteins is about 7%. Asparagine synthase can convert aspartate to the polar amino acid asparagine. Aspartate is also a precursor for cellular signaling compounds such as, N-acetyl-aspartate, beta-alanine, adenylsuccinate, arginino-succinate and N-carbamoylaspartate. Aspartate is also a metabolite in the urea cycle and involved in gluconeogenesis. Additionally, aspartate carries the reducing equivalents in the mitochondrial malate-aspartate shuttle, which utilizes the ready interconversion of aspartate and oxaloacetate. The conjugate base of L-aspartic acid, aspartate, also acts as an excitatory neurotransmitter in the brain which activates NMDA receptors.
|
|
|
|
SMP0000066 |
Biotin MetabolismBiotin is a vitamin that is an essential nutrient for humans. Biotin can be absorbed from consuming various foods such as: legumes, soybeans, tomatoes, romaine lettuce, eggs, cow's milk, oats and many more. Biotin acts as a cofactor for enzymes to catalyze carboxylation reactions involved in gluconeogenesis, amino acid catabolism and fatty acid metabolism. Biotin deficiency has been associated with many human diseases. These diseases may be caused by dysfunctional biotin metabolism due to enzyme deficiencies. Some research suggests biotin may play a role in transcription regulation or protein expression which may lead to biotin related diseases.
|
|||
SMP0000065 |
Ubiquinone BiosynthesisUbiquinone is also known as coenzyme Q10. It is a 1,4-benzoquinone, where Q refers to the quinone chemical group, and 10 refers to the isoprenyl chemical subunits. Ubiquinone is a carrier of hydrogen atoms (protons plus electrons) and functions as an ubiquitous coenzyme in redox reactions, where it is first reduced to the enzyme-bound intermediate radical semiquinone and in a second reduction to ubiquinol (Dihydroquinone; CoQH2). Ubiquinone is not tightly bound or covalently linked to any known protein complex but is very mobile. In eukaryotes ubiquinones were found in the inner mito-chondrial membrane and in other membranes such as the endoplasmic reticulum, Golgi vesicles, lysosomes and peroxisomes. The benzoquinone portion of Coenzyme Q10 is synthesized from tyrosine, whereas the isoprene sidechain is synthesized from acetyl-CoA through the mevalonate pathway. The mevalonate pathway is also used for the first steps of cholesterol biosynthesis. The enzyme para-hydroxybenzoate polyprenyltransferase catalyzes the condensation of p-hydroxybenzoate with polyprenyl diphosphate to generate ubiquinone.
|
|
||
SMP0000064 |
Fructose and Mannose DegradationFructose and mannose are monosaccharides that can be found in many foods. Fructose can join with glucose to form sucrose. Mannose can be converted to glucose. Both may be used as food sweeteners. Fructose is well absorbed, especially in the presence of glucose. Fructose causes less of an insulin response compared to glucose and thus may be a preferred sugar for diabetics. In contrast to fructose, humans do not metabolize mannose well with the majority of it being excreted unchanged. Mannose in the urine can be beneficial in treating urinary tract infections caused be E. coli. However, mannose can be detrimental to humans by causing diabetic complications.
|
|
|
|
SMP0000063 |
Tryptophan MetabolismThis pathway depicts the metabolic reactions and pathways associated with tryptophan metabolism in animals. Tryptophan is an essential amino acid. This means that it cannot be synthesized by humans and other mammals and therefore must be part of the diet. Unlike animals, plants and microbes can synthesize tryptophan from shikimic acid or anthranilate. As one of the 20 proteogenic amino acids, tryptophan plays an important role in protein biosynthesis through the action of tryptophanyl-tRNA synthetase. As shown in this pathway, tryptophan can be linked to the tryptophanyl-tRNA via either the mitochondrial or cytoplasmic tryptophan tRNA ligases. Also shown in this pathway map is the conversion of tryptophan to serotonin (a neurotransmitter). In this process, tryptophan is acted upon by the enzyme tryptophan hydroxylase, which produces 5-hydroxytryptophan (5HTP). 5HTP is then converted into serotonin (5-HT) via aromatic amino acid decarboxylase. Serotonin, in turn, can be converted into N-acetyl serotonin (via serotonin-N-acetyltransferase) and then melatonin (a neurohormone), via 5-hydroxyindole-O-methyltransferase. The melatonin can be converted into 6-hydroxymelatonin via the action of cytochrome P450s in the endoplasmic reticulum. Serotonin has other fates as well. As depicted in this pathway it can be converted into N-methylserotonin via Indolethylamine-N-methyltransferase (INMT) or it can be converted into formyl-5-hydroxykynurenamine via indoleamine 2,3-dioxygenase. Serotonin may also be converted into 5-methoxyindoleacetate via a series of intermediates including 5-hydroxyindoleacetaldehyde and 5-hydroxyindoleacetic acid. Tryptophan can be converted or broken down into many other compounds as well. It can be converted into tryptamine via the action of aromatic amino acid decarboxylase. The resulting tryptamine can then be converted into indoleacetaldehyde via kynurenine 3-monooxygenase and then into indoleacetic acid via the action of aldehyde dehydrogenase. Tryptophan also leads to the production of a very important compound known as kynurenine. Kynurenine is synthesized via the action of tryptophan 2,3-dioxygnase, which produces N-formylkynurenine. This compound is converted into kynurenine via the enzyme known as kynurenine formamidase (AFMID). Kynurenine has at least 3 fates. First, kynurenine can undergo deamination in a standard transamination reaction yielding kynurenic acid. Secondly, kynurenine can undergo a series of catabolic reactions (involving kynureninase and kynurenine 3-monooxygenase) producing 3-hydroxyanthranilate plus alanine. In this reaction, kynureninase catabolizes the conversion of kynurenine into anthranilic acid while kynurenine—oxoglutarate transaminase (also known as kynurenine aminotransferase or glutamine transaminase K, GTK) catabolizes its conversion into kynurenic acid. The action of kynurenine 3-hydroxylase on kynurenic acid leads to 3-hydroxykynurenine. The oxidation of 3-hydroxyanthranilate converts it into 2-amino-3-carboxymuconic 6-semialdehyde, which has two fates. It can either degrade to form acetoacetate or it can cyclize to form quinolate. Most of the body’s 3-hydroxyanthranilate leads to the production of acetoacetate (a ketone body), which is why tryptophan is also known as a ketogenic amino acid. An important side reaction in the liver involves a non-enzymatic cyclization into quinolate followed by transamination and several rearrangements to yield limited amounts of nicotinic acid, which leads to the production of a small amount of NAD+ and NADP+.
|
|
|
|
SMP0000060 |
Pyruvate MetabolismPyruvate is an intermediate compound in the metabolism of fats, proteins, and carbohydrates. It can be formed from glucose via glycolysis or the transamination of alanine. It can be converted into Acetyl-CoA to be used as the primary energy source for the TCA cycle, or converted into oxaloacetate to replenish TCA cycle intermediates. Pyruvate can also be used to synthesize carbohydrates, fatty acids, ketone bodies, alanine, and steroids. In conditions of inssuficient oxygen or in cells with few mitochondria, pyruvate is reduced to lactate in order to re-oxidize NADH back into NAD+
Pyruvate participates in several key reactions and pathways. In glycolysis, phosphoenolpyruvate (PEP) is converted to pyruvate by pyruvate kinase in an highly exergonic and irreversible reaction. In gluconeogenesis, pyruvate carboxylase and PEP carboxykinase are needed to catalyze the conversion of pyruvate to PEP. In fatty acid synthesis, the pyruvate dehydrogenase complex decarboxylates pyruvate to produce acetyl-CoA. In gluconeogenesis, the carboxylation by pyruvate carboxylase produces oxaloacetate. The fate of pyruvate depends on the cell energy charge. In cells or tissues with a high energy charge pyruvate is directed toward gluconeogenesis, but when the energy charge is low pyruvate is preferentially oxidized to CO2 and H2O in the TCA cycle, with generation of 15 equivalents of ATP per pyruvate. The enzymatic activities of the TCA cycle are located in the mitochondrion. When transported into the mitochondrion, pyruvate encounters two principal metabolizing enzymes: pyruvate carboxylase (a gluconeogenic enzyme) and pyruvate dehydrogenase (PDH). With a high cell-energy charge, acetyl-CoA, is able allosterically to activate pyruvate carboxylase, directing pyruvate toward gluconeogenesis. When the energy charge is low CoA is not acylated, pyruvate carboxylase is inactive, and pyruvate is preferentially metabolized via the PDH complex and the enzymes of the TCA cycle to CO2 and H2O.
|
|
|
|
SMP0000059 |
Urea CycleUrea, also known as carbamide, is a waste product made by a large variety of living organisms and is the main component of urine. Urea is created in the liver, through a string of reactions that are called the Urea Cycle. This cycle is also
called the Ornithine Cycle, as well as the Krebs-Henseleit Cycle. There are some essential compounds required for the completion of this cycle, such as arginine, citrulline and ornithine. Arginine cleaves and creates urea and ornithine, and the reactions that follow see urea residue build up on ornithine, which recreates arginine and keeps the cycle going. Ornithine is transported to the mitochondrial matrix, and once there, ornithine carbamoyltransferase uses carbamoyl phosphate to create citrulline. After this, citrulline is transported to the cytosol. Once here, citrulline and aspartate team up to create argininosuccinic acid. After this, argininosuccinate lyase creates l-arginine. L-arginine finally uses arginase-1 to create ornithine again, which will be transported to the mitochondrial matrix and restart the urea cycle once more.
|
|
|
|
SMP0000058 |
Starch and Sucrose MetabolismAmylase enzymes secreted in saliva by the parotid gland and in the small intestine play an important role in initiating starch digestion. The products of starch digestion are but not limited to maltotriose, maltose, limit dextrin, and glucose. The action of enterocytes of the small intestine microvilli further break down limit dextrins and disaccharides into monosaccharides: glucose, galactose, and fructose. Once released from starch or once ingested, sucrose can be degraded into beta-D-fructose and alpha-D-glucose via lysosomal alpha-glucosidase or sucrose-isomaltase. Beta-D-fructose can be converted to beta-D-fructose-6-phosphate by glucokinase and then to alpha-D-glucose-6-phosphate by the action of glucose phosphate isomerase. Phosphoglucomutase 1 can then act on alpha-D-glucose-6-phosphate (G6P) to generate alpha-D-glucose-1-phosphate. Alpha-D-glucose-1-phosphate (G6P) has several possible fates. It can enter into gluconeogenesis, glycolysis or the nucleotide sugar metabolism pathway. UDP-glucose pyrophosphorylase 2 can convert alpha-D-glucose-1-phosphate into UDP-glucose, which can then be converted to UDP-xylose or UDP-glucuronate and, eventually to glucuronate. UDP-glucose can also serve as a precursor to the synthesis of glycogen via glycogen synthase. Glycogen is an analogue of amylopectin (“plant starch”) and acts as a secondary short-term energy storage for animal cells. It’s formed primarily in liver and muscle tissues, but is also formed at secondary sites such as the central nervous system and the stomach. In both cases it exists as free granules in the cytosol. Glycogen is a crucial element of the glucose cycle as another enzyme, glycogen phosphorylase, cleaves off glycogen from the nonreducing ends of a chain to producer glucose-1-phosphate monomers. From there, the glucose-1-phosphate monomers have three possible fates: (1) enter the glycolysis pathway as glucose-6—phosphate (G6P) to generate energy, (2) enter the pentose phosphate pathway to produce NADPH and pentose sugar, or (3) enter the gluconeogenesis pathway by being dephosphorylated into glucose in liver or kidney tissues.
To initiate the process of glycogen chain-lengthening, glycogenin is required because glycogen synthase can only add to existing chains. This action is subsequently followed by the action of glycogen synthase which catalyzes the formation of polymers of UDP-glucose connected by (α1→4) glycosidic bonds to form a glycogen chain. Importantly, amylo (α1→4) to (α1→6) transglycosylase catalyzes glycogen branch formation via the transfer of 6-7 glucose residues from a nonreducing end with greater than 11 residues to the C-6 OH- group in the interior of a glycogen molecule.
|
|
|
|
SMP0000057 |
Citric Acid CycleThe citric acid cycle, which is also known as the tricarboxylic acid cycle (TCA cycle) or the Krebs cycle, is a connected series of enzyme-catalyzed chemical reactions of central importance to all aerobic organisms (i.e. organisms that use oxygen for cellular respiration). The citric acid cycle is named after citrate or citric acid, a tricarboxylic acid that is both consumed and regenerated through this pathway. The citric acid cycle was discovered in 1937 by Hans Adolf Krebs while he worked at the University of Sheffield in England (PMID: 16746382). Krebs received the Nobel Prize for his discovery in 1953. Krebs’ extensive work on this pathway is also why the citric acid or TCA cycle is often referred to as the Krebs cycle. Metabolically, the citric acid cycle allows the release of energy (ultimately in the form of ATP) from carbohydrates, fats, and proteins through the oxidation of acetyl-CoA. The citric acid cycle also produces CO2, the precursors for several amino acids (aspartate, asparagine, glutamine, proline) and NADH – all of which are used in other important metabolic pathways, such as amino acid synthesis and oxidative phosphorylation (OxPhos). The net yield of one “turn” of the TCA cycle in terms of energy-containing compounds is one GTP, one FADH2, and three NADH molecules. The NADH molecules are used in oxidative phosphorylation to generate ATP. In eukaryotes, the citric acid cycle occurs in the mitochondrial matrix. In prokaryotes, the citric acid cycle occurs in the cytoplasm. In eukaryotes, the citric acid or TCA cycle has a total of 10 steps that are mediated by 8 different enzymes. Key to the whole cycle is the availability of acetyl-CoA. One of the primary sources of acetyl-CoA is from the breakdown of glucose (and other sugars) by glycolysis. This process generates pyruvate. Pyruvate is decarboxylated by pyruvate dehydrogenase to generate acetyl-CoA. The citric acid cycle begins with acetyl-CoA transferring its two-carbon acetyl group to the four-carbon acceptor compound (oxaloacetate) to form a six-carbon compound (citrate) through the enzyme citrate synthase. The resulting citrate is then converted to cis-aconitate and then isocitrate via the enzyme aconitase. The resulting isocitrate then combines with NAD+ to form oxalosuccinate and NADH, which is then converted into alpha-ketoglutarate (and CO2) through the action of the enzyme known as isocitrate dehydrogenase. The resulting alpha-ketoglutarate combines with NAD+ and CoA-SH to produce succinyl-CoA, NADH, and CO2. This step is mediated by the enzyme alpha-ketoglutarate dehydrogenase. The resulting succinyl-CoA combines with GDP and organic phosphate to produce succinate, CoA-SH, and GTP. This phosphorylation reaction is performed by succinyl-CoA synthase. The resulting succinate then combines with ubiquinone to produce two compounds, fumarate and ubiquinol through the action of the enzyme succinate dehydrogenase. The resulting fumarate is then hydrated by the enzyme known as fumarase to produce malate. The resulting malate is oxidized via NAD+ to produce oxaloacetate and NADH. This oxidation reaction is performed by malate dehydrogenase. The resulting oxaloacetate can then combine with acetyl-CoA and the TCA reaction cycle begins again. Overall, in the citric acid cycle, the starting six-carbon citrate molecule loses two carboxyl groups as CO2, leading to the production of a four-carbon oxaloacetate. The two-carbon acetyl-CoA that is the “fuel” for the TCA cycle can be generated by several metabolic pathways including glucose metabolism, fatty acid oxidation, and the metabolism of amino acids. The overall reaction for the citric acid cycle is as follows: acetyl-CoA + 3 NAD+ + FAD + GDP + P + 2H2O = CoA-SH + 3NADH + FADH2 + 3H+ + GTP + 2CO2. Many molecules in the citric acid cycle serve as key precursors for other molecules needed by cells. The citrate generated via the citric acid cycle can serve as an intermediate for fatty acid synthesis; alpha-ketoglutarate can serve as a precursor for glutamate, proline, and arginine; oxaloacetate can serve as a precursor for aspartate and asparagine; succinyl-CoA can serve as a precursor for porphyrins; and acetyl-CoA can serve as a precursor fatty acids, cholesterol, vitamin D, and various steroid hormones. There are several variations to the citric acid cycle that are known. Interestingly, most of the variation lies with the step involving succinyl-CoA production or conversion. Humans and other animals have two different types of succinyl-CoA synthetases. One produces GTP from GDP, while the other produces ATP from ADP (PMID: 9765291). On the other hand, plants have a succinyl-CoA synthetase that produces ATP (ADP-forming succinyl-CoA synthetase) (Jones RC, Buchanan BB, Gruissem W. (2000). Biochemistry & molecular biology of plants (1st ed.). Rockville, Md: American Society of Plant Physiologists. ISBN 0-943088-39-9.). In certain acetate-producing bacteria, such as Acetobacter aceti, an enzyme known as succinyl-CoA:acetate CoA-transferase performs this conversion (PMID: 18502856) while in Helicobacter pylori succinyl-CoA:acetoacetate CoA-transferase is responsible for this reaction (PMID: 9325289). The citric acid cycle is regulated in a number of ways but the primary mechanism is by product inhibition. For instance, NADH inhibits pyruvate dehydrogenase, isocitrate dehydrogenase, alpha-ketoglutarate dehydrogenase, and citrate synthase. Acetyl-CoA inhibits pyruvate dehydrogenase, while succinyl-CoA inhibits alpha-ketoglutarate dehydrogenase and citrate synthase. Additionally, ATP inhibits citrate synthase and alpha-ketoglutarate dehydrogenase. Calcium is another important regulator of the citric acid cycle. In particular, it activates pyruvate dehydrogenase phosphatase, which then activates pyruvate dehydrogenase. Calcium also activates isocitrate dehydrogenase and alpha-ketoglutarate dehydrogenase (PMID: 171557).
|
|
|
|
SMP0000055 |
Alanine MetabolismAlanine (L-Alanine) is an α-amino acid that is used for protein biosynthesis. Approximately 8% of human proteins have alanine in their structures. The reductive lamination of pyruvate is effected by alanine transaminase. L-Alanine can be converted to pyruvic acid by alanine aminotransferase 1 reversibly coupled with interconversion of oxoglutaric acid and L-glutamic acid. L-Alanine can also be produced by alanine-glyoxylate transaminase with coupled interconversion of glyoxylate and glycine. L-Alanine will be coupled with alanyl tRNA by alanyl-tRNA synthetase to perform protein biosynthesis. Alanine can also be used to provide energy under fasting conditions. There are two pathways that can facilitate this: (1) alanine is converted to pyruvate to synthesize glucose via the gluconeogenesis pathway in liver tissue or (2) alanine converted into pyruvate moves into the TCA cycle to be oxidized in other tissues.
|
Showing 64951 -
64960 of 65006 pathways