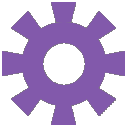
Browsing Pathways
Showing 64971 -
64980 of 605359 pathways
SMPDB ID | Pathway Name and Description | Pathway Class | Chemical Compounds | Proteins |
---|---|---|---|---|
SMP0000041 |
Sulfate/Sulfite MetabolismThis pathway illustrates the conversion of sulfite to sulfate (via sulfate oxidase) and subsequent generation of adenylylsulfate (APS) via 3'-phosphoadenosine 5'-phosphosulfate synthase 2. APS is converted to phosphoadenylyl-sulfate (PAPS) via adenylylsulfate kinase. APS can also be regenerated from PAPS by 3'(2'), 5'-bisphosphate nucleotidase 1. PAPS is eventually converted to adenosine bisophosphate (PAP) through the action of several different enzymes including aryl sulfotransferase, chondroitin 4-sulfotransferase 13 and estrone sulfotransferase.
The metabolism pathway in question is important for many reasons. Recall, that the sulfite ion is in fact the conjugate base of sulfurous acid. Moreover, this ion is found naturally in one of the worlds most popular beverages, wines. Beyond its natural occurence, sulfite ion had the property of stopping fermentation. As such, the addition of it to products such as wine can be used either as a preservative or to stop the fermentation process at a moment which is of interest. Finally, this preservation property goes beyond merely wines, and finds utility in dried fruits, potatoes, etc.
|
|
||
SMP0000040 |
GlycolysisGlycolysis is a metabolic pathway with sequence of ten reactions involving ten intermediate compounds that converts glucose to pyruvate. Glycolysis release free energy for forming high energy compound such as ATP and NADH. Glycolysis is consisted of two phases, which one of them is chemical priming phase and second phase is energy-yielding phase. As the starting compound of chemical priming phase, D-glucose can be obtained from galactose metabolism or imported by monosaccharide-sensing protein 1 from outside of cell. D-Glucose is catalyzed by probable hexokinase-like 2 protein to form glucose 6-phosphate which is powered by ATP. Glucose 6-phosphate transformed to fructose 6-phosphate by glucose-6-phosphate isomerase, which the later compound will be converted to fructose 1,6-bisphosphate, which is the last reaction of chemical priming phase by 6-phosphofructokinase with cofactor magnesium, and it is also powered by ATP. Before entering the second phase, aldolase catalyzing the hydrolysis of F1,6BP into dihydroxyacetone phosphate and glyceraldehyde 3-phosphate. Dihydroxyacetone phosphate and glyceraldehyde 3-phosphate can convert to each other bidirectionally by facilitation of triosephosphate isomerase. The second phase of glycolysis is yielding-energy phase that produce ATP and NADH. At the first step, D-glyceraldehyde 3-phosphate is catalyzed to glyceric acid 1,3-biphosphate by glyceraldehyde-3-phosphate dehydrogenase with NAD, which also generate NADH. ATP is generated through the reaction that convert glyceric acid 1,3-biphosphate to 3-phosphoglyceric acid. Phosphoglycerate mutase 2 catalyze 3-phosphoglyceric acid to 2-Phospho-D-glyceric acid, and alpha-enolase with cofactor magnesium catalyzes 2-Phospho-D-glyceric acid to phosphoenolpyruvic acid. Eventually, plastidial pyruvate kinase 4 converts phosphoenolpyruvic acid to pyruvate with cofactor magnesium and potassium and ADP. Pyruvate will undergo pyruvate metabolism, tyrosine metabolism and pantothenate and CoA biosynthesis.
|
|
|
|
SMP0000039 |
Glycerolipid MetabolismThe glycerolipid metabolism pathway describes the synthesis of glycerolipids such as monoacylglycerols (MAGs), diacylglycerols (DAGs), triacylglycerols (TAGs), phosphatidic acids (PAs), and lysophosphatidic acids (LPAs). The process begins with cytoplasmic 3-phosphoglyceric acid (a product of glycolysis). This molecule is dephosphorylated via the enzyme glycerate kinase to produce glyceric acid. Glyceric acid is then transformed to glycerol (via the action of aldehyde dehydrogenase and aldose reductase). The free, cytoplasmic glycerol can then be phosphorylated to glycerol-3-phosphate through the action of glycerol kinase. Glycerol-3-phosphate can then enter the endoplasmic reticulum where glycerol-3-phosphate acyltransferase (GPAT) may combine various acyl-CoA moieties (which donate acyl groups) to form lysophosphatidic (LPA) or phosphatidic acid (PA). The resulting phosphatidic acids can be dephosphorylated via lipid phosphate phosphohydrolase (also known as phosphatidate phosphatase) to produce diacylglycerols (DAGs). The resulting DAGs can be converted into triacylglycerols (TAGs) via the addition of another acyl group (contributed via acyl-CoA) and the action of 1-acyl-sn-glycerol-3-phosphate acyltransferase. Extracellularly, the triacylglycerols (TAGs) can be converted to monoacylglycerols (MAGs) through the action of hepatic triacylglycerol lipase. In addition to this cytoplasmic route of glycerolipid synthesis, another route via mitochondrial synthesis also exists. This route begins with glycerol-3-phosphate, which can be either derived from dihydroxyacetone phosphate (DHAP), a product of glycolysis (usually in the cytoplasm of liver or adipose tissue cells) or from glycerol itself. Glycerol-3-phosphate in the mitochondria is first acylated via acyl-coenzyme A (acyl-CoA) through the action of mitochondrial glycerol-3-phosphate acyltransferase to form lysophosphatidic acid (LPA). Once synthesized, lysophosphatidic acid is then acylated with another molecule of acyl-CoA via the action of 1-acyl-sn-glycerol-3-phosphate acetyltransferase to yield phosphatidic acid. Phosphatidic acid is then dephosphorylated to form diacylglycerol. Specifically, diacylglycerol is formed by the action of phosphatidate phosphatase (also known as lipid phosphate phosphohydrolase) on phosphatidic acid coupled with the release of a phosphate. The phosphatase exists as 3 isozymes. Diacylglycerol is a precursor to triacylglycerol (triglyceride), which is formed in the addition of a third fatty acid to the diacylglycerol by the action of diglyceride acyltransferase. Since diacylglycerol is synthesized via phosphatidic acid, it will usually contain a saturated fatty acid at the C-1 position on the glycerol moiety and an unsaturated fatty acid at the C-2 position. When the body uses stored fat as a source of energy, glycerol and fatty acids are released into the bloodstream. Fatty acids, stored as triglycerides in humans, are an important and a particularly rich source of energy. The energy yield from a gram of fatty acids is approximately 9 kcal/g (39 kJ/g), compared to 4 kcal/g (17 kJ/g) for carbohydrates. Since the hydrocarbon portion of fatty acids is hydrophobic, these molecules can be stored in a relatively anhydrous (water-free) environment. Fatty acids can hold more than six times the amount of energy than sugars on a weight basis. In other words, if you relied on sugars or carbohydrates to store energy, then you would need to carry 67.5 lb (31 kg) of glycogen to have the energy equivalent to 10 lb (5 kg) of fat.
|
|
|
|
SMP0000037 |
Lysine DegradationThe degradation of L-lysine happens in liver and it is consisted of seven reactions. L-Lysine is imported into liver through low affinity cationic amino acid transporter 2 (cationic amino acid transporter 2/SLC7A2). Afterwards, L-lysine is imported into mitochondria via mitochondrial ornithine transporter 2. L-Lysine can also be obtained from biotin metabolism. L-Lysine and oxoglutaric acid will be combined to form saccharopine by facilitation of mitochondrial alpha-aminoadipic semialdehyde synthase, and then, mitochondrial alpha-aminoadipic semialdehyde synthase will further breaks saccharopine down to allysine and glutamic acid. Allysine will be degraded to form aminoadipic acid through alpha-aminoadipic semialdehyde dehydrogenase. Oxoadipic acid is formed from catalyzation of mitochondrial kynurenine/alpha-aminoadipate aminotransferase on aminoadipic acid. Oxoadipic acid will be further catalyzed to form glutaryl-CoA, and glutaryl-CoA converts to crotonoyl-CoA, and crotonoyl-CoA transformed to 3-hydroxybutyryl-CoA. 3-Hydroxybutyryl-CoA will form Acetyl-CoA as the final product through the intermediate compound: acetoacetyl-CoA. Acetyl-CoA will undergo citric acid cycle metabolism. Carnitine is another key byproduct of lysine metabolism (not shown in this pathway).
|
|
|
|
SMP0000036 |
D-Arginine and D-Ornithine MetabolismD-Amino acids have been show to be present in high concentrations in humans and play a role in biological functions. D-Amino may have negative effects as they can be found in some bacteria or form spontaneously in certain reactions. D-Amino acid oxidase (DAAO) is one of the main enzymes that metabolize D-Amino acids via deamination. DAAO is highly specific towards D-amino acids and favours free neutral D-amino acids or those with hydrophobic, polar or aromatic groups. Acidic amino acids are not catalyze by DAOO.
|
|||
SMP0000035 |
Bile Acid BiosynthesisA bile acids life begins as cholesterol is catabolized, as bile acid is a derivative of cholesterol. This pathway occurs in the liver, beginning with cholesterol being converted to 7a-hydroxycholesterol through the enzyme cholesterol-7-alpha-monooxygenase, after being transported into the liver cell. 7a-hydroxycholesterol then becomes 7a-hydroxy-cholestene-3-one, which is made possible by the enzyme 3-beta-hydroxysteroid dehydrogenase type 7. 7a-hydroxy-cholestene-3-one then is used in two different chains of reactions. The first, continuing in the liver, uses the enzyme 3-oxo-5-beta-steroid-4-deydrogenase to become 7a-hydroxy-5b-cholestan-3-one. After that, aldo-keto reductase family 1 member C4 is used to create 3a,7a-dihydroxy-5b-cholestane. In the mitochondria of the cell, sterol 26-hydroxylase converts 3a,7a-dihydroxy-5b-cholestane to 3a,7a,26-trihydroxy-5b-cholestane, which is then converted to 3a,7a-dihydroxy-5b-cholestan-26-al by the same enzyme used in the previous reaction. This enzyme is used another time, to create 3a,7a-dihydroxycoprostanic acid. Then, bile acyl-CoA synthetase teams up with 3a,7a-dihydroxycoprostanic acid to create 3a,7a-dihydroxy-5b-cholestanoyl-CoA. 3a,7a-dihydroxy-5b-cholestanoyl-CoA remains intact while alpha-methylacyl-CoA racemase moves it along through the peroxisome. Peroxisomal acyl coenzyme A oxidase 2 converts 3a,7a-dihydroxy-5b-cholestanoyl-CoA into 3a,7a-dihydoxy-5b-cholest-24-enoyl-CoA. With the help of water, peroxisomal multifunctional enzyme type 2 turns 3a,7a-dihydoxy-5b-cholest-24-enoyl-CoA into 3a,7a,24-trihydoxy-5b-cholestanoyl-CoA. This compound then uses peroxisomal multifunctional enzyme type 2 to create chenodeoxycholoyl-CoA. From there, propionyl-CoA and chenodeoxycholoyl-CoA join forces and enlist the help of non-specific lipid transfer protein to further chenodeoxycholoyl-CoAâ€TMs journey in the peroxisome. It is then transported back into intracellular space, where after its used in 3 different reactions, its derivatives interact with intestinal microflora in the extracellular space to become lithocholyltaurine, lithocholic acid glycine conjugate, and lithocholic acid. Revisiting 7a-hydroxy-cholestene-3-one, the second chain of reactions it is involved in follows a similar path as the first, moving through the mitochondria, endoplasmic reticulum and peroxisome until choloyl-CoA is formed, which then is used in three reactions so that its derivatives may leave the cell to interact with intestinal microflora and become taurodeoxycholic acid, deoxycholic acid glycine conjugate and deoxycholic acid. There are two more important components of this pathway, both depicting the breakdown of cholesterol into bile acid. These components of the pathway occur in the endoplasmic reticulum membrane, although 2 enzymes, 25-hydroxycholesterol 7-alpha-hydroxylase and sterol 26 hydroxylase, are found in the mitochondria. Bile acids play a very important part in the digestion of foods, and are responsible for the absorption of water soluble vitamins in the small intestine. Bile acids also help absorb fats into the small intestine, a crucial part of any vertebrates diet.
|
|
|
|
SMP0000034 |
Sphingolipid MetabolismThe sphingolipid metabolism pathway depicted here describes the synthesis of sphingolipids which include sphingomyelins, ceramides, phosphoceramides, glucosylceramides, galactosylceramides, sulfagalactosylceramides, lactosylceramides, and various other ceramides. The core of a sphingolipid is the long-chain amino alcohol called sphingosine. Amino acylation, with a long-chain fatty acid, at the 2-carbon position of sphingosine yields a ceramide. Sphingolipids are a component of all membranes but are particularly abundant in the myelin sheath. De novo sphingolipid synthesis begins at the cytoplasmic side of the ER (endoplasmic reticulum) with the formation of 3-keto-dihydrosphingosine (also known as 3-ketosphinganine) by the enzyme known as serine palmitoyltransferase (SPT). The preferred substrates for this reaction are palmitoyl-CoA and serine. Next, 3-keto-dihydrosphingosine is reduced to form dihydrosphingosine (also known as sphinganine) via the enzyme 3-ketodihydrosphingosine reductase (KDHR), which is also known as 3-ketosphinganine reductase. Dihydrosphingosine (sphinganine) is acylated by the action of several dihydroceramide synthases (CerS) to form dihydroceramide. Dihydroceramide is then desaturated in the original palmitic portion of the lipid via dihydroceramide desaturase 1 (DES1) to form ceramide. Following the conversion to ceramide, sphingosine is released via the action of ceramidase. Sphingosine can be re-converted into a ceramide by condensation with an acyl-CoA catalyzed by the various CerS enzymes. Ceramide may be phosphorylated by ceramide kinase to form ceramide-1-phosphate. Alternatively, it may be glycosylated by glucosylceramide synthase (to form a glucosylceramide) or galactosylceramide synthase (to form a galactosylceramide). Additionally, it can be converted to sphingomyelin by the addition of a phosphorylcholine headgroup by sphingomyelin synthase (SMS). Sphingomyelins are the only sphingolipids that are phospholipids. Diacylglycerol is also generated via this process. Alternately, ceramide may be broken down by a ceramidase to form sphingosine. Sphingosine may be phosphorylated to form sphingosine-1-phosphate, which may, in turn, be dephosphorylated to regenerate sphingosine. Sphingolipid catabolism allows the reversion of these metabolites to ceramide. The complex glycosphingolipids are hydrolyzed to glucosylceramide and galactosylceramide. These lipids are then hydrolyzed by beta-glucosidases and beta-galactosidases to regenerate ceramide. Similarly, sphingomyelins may be broken down by sphingomyelinase to create ceramides and phosphocholine. The only route by which sphingolipids are converted into non-sphingolipids is through sphingosine-1-phosphate lyase. This forms ethanolamine phosphate and hexadecenal.
|
|
|
|
SMP0000033 |
Methionine MetabolismMethionine metabolism is a process that is necessary for humans. Methionine metabolism in mammals happens within two pathways, a methionine cycle and a transsulfuration sequence. These pathways have three common reactions with both pathways including the transformation of methionine to S-adenosylmethionine (SAM), the use of SAM in many different transmethylation reactions resulting in a methylated product plus S-adenosylhomocysteine, and the conversion of S-adenosylhomocysteine to produce the compounds homocysteine and adenosine. The reactions mentioned above not only produce cysteine, they also create a-ketobutyrate. This compound is then converted to succinyl-CoA through a three step process after being converted to propionyl-CoA. If the amino acids cysteine and methionine are available in enough quantity, the pathway will accumulate SAM and this will in turn encourage the production of cysteine and a-ketobutyrate, which are both glucogenic, through cystathionine synthase. When there is a lack of methionine, there is a decrease in the production of SAM, which limits cystathionine synthase activity.
|
|
|
|
SMP0000032 |
Valine, Leucine, and Isoleucine DegradationValine, isoleuciine, and leucine are essential amino acids and are identified as the branched-chain amino acids (BCAAs). The catabolism of all three amino acids starts in muscle and yields NADH and FADH2 which can be utilized for ATP generation. The catabolism of all three of these amino acids uses the same enzymes in the first two steps. The first step in each case is a transamination using a single BCAA aminotransferase, with α-ketoglutarate as the amine acceptor. As a result, three different α-keto acids are produced and are oxidized using a common branched-chain α-keto acid dehydrogenase (BCKD), yielding the three different CoA derivatives. Isovaleryl-CoA is produced from leucine by these two reactions, alpha-methylbutyryl-CoA from isoleucine, and isobutyryl-CoA from valine. These acyl-CoA’s undergo dehydrogenation, catalyzed by three different but related enzymes, and the breakdown pathways then diverge. Leucine is ultimately converted into acetyl-CoA and acetoacetate; isoleucine into acetyl-CoA and succinyl-CoA; and valine into propionyl-CoA (and subsequently succinyl-CoA). Under fasting conditions, substantial amounts of all three amino acids are generated by protein breakdown. In muscle, the final products of leucine, isoleucine, and valine catabolism can be fully oxidized via the citric acid cycle; in the liver, they can be directed toward the synthesis of ketone bodies (acetoacetate and acetyl-CoA) and glucose (succinyl-CoA). Because isoleucine catabolism terminates with the production of acetyl-CoA and propionyl-CoA, it is both glucogenic and ketogenic. Because leucine gives rise to acetyl-CoA and acetoacetyl-CoA, it is classified as strictly ketogenic.
|
|
|
|
SMP0000031 |
Pentose Phosphate PathwayThe pentose phosphate pathway—also referred to in the literature as the phosphogluconate pathway, the hexose monophosphate shunt, or the pentose phosphate shunt—is involved in the generation of NADPH as well as pentose sugars. Of the total cytoplasmic NADPH used in biosynthetic reactions, a significant proportion of it is generated through the pentose phosphate pathway. Ribose 5-phosphate is also another essential product generated by this pathway which is employed in nucleotide synthesis. The pentose phosphate pathway is also involved in the digestive process as the products of nucleic acid catabolism can be metabolized through the pathway (pentose sugars are usually yielded in the breakdown) while the carbon backbones of dietary carbohydrates can be converted into glycolytic/gluconeogenic intermediates. The pentose phosphate pathway is interconnected to the glycolysis pathway through the shared use of three intermediates: glucose 6-phosphate, glyceraldehyde 3-phosphate, and fructose 6-phosphate.
The pathway can be described as eight distinct reactions (see below) and is separated into an oxidative phase and a non-oxidative phase. Reactions 1-3 form the oxidative phase and generate NADPH and pentose 5-phosphate. Reactions 4-8 form the non-oxidative phase and converts pentose 5-phosphate into other pentose sugars such as ribose 5-phosphate, but generates no NADPH. The eight reactions are as follows: reaction 1 where glucose-6-phosphate 1-dehydrogenase converts glucose 6-phosphate into D-glucono-1,5-lactone 6-phosphate with NADPH formation; reaction 2 where 6-phosphogluconolactonase converts D-glucono-1,5-lactone 6-phosphate into 6-phospho-D-gluconate;reaction 3 where 6-phosophogluconate dehydrogenase converts 6-phospho-D-gluconate into ribulose 5-phosphate with NADPH formation; reaction 4 where ribulose-phosphate 3-epimerase converts ribulose 5-phosphate into xylulose 5-phosphate; reaction 5 where ribose-5-phosphate isomerase converts ribulose 5-phosphate into ribose 5-phosphate; reaction 6 where transketolase rearranges ribose 5-phosphate and xylulose 5-phosphate to form sedoheptulose 7-phosphate and glyceraldehyde 3-phosphate; reaction 7 where transaldolase rearranges of sedoheptulose 7-phosphate and glyceraldehyde 3-phosphate to form erythrose 4-phosphate and fructose 6-phosphate; and reaction 8 where transkelotase rearranges of xylulose 5-phosphate and erythrose 4-phosphate to form glyceraldehyde 3-phosphate and fructose-6-phosphate.
|
|
|
Showing 64971 -
64980 of 65006 pathways