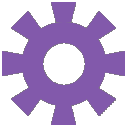
Browsing Pathways
Showing 101 -
110 of 605359 pathways
SMPDB ID | Pathway Name and Description | Pathway Class | Chemical Compounds | Proteins |
---|---|---|---|---|
SMP0000452 |
Threonine and 2-Oxobutanoate Degradation 2-oxobutanoate, also known as 2-Ketobutyric acid, is a 2-keto acid that is commonly produced in the metabolism of amino acids such as methionine and threonine. Like other 2-keto acids, degradation of 2-oxobutanoate occurs in the mitochondrial matrix and begins with oxidative decarboxylation to its acyl coenzyme A derivative, propionyl-CoA. This reaction is mediated by a class of large, multienzyme complexes called 2-oxo acid dehydrogenase complexes. While no 2-oxo acid dehydrogenase complex is specific to 2-oxobutanoate, numerous complexes can catalyze its reaction. In this pathway the branched-chain alpha-keto acid dehydrogenase complex is depicted. All 2-oxo acid dehydrogenase complexes consist of three main components: a 2-oxo acid dehydrogenase (E1) with a thiamine pyrophosphate cofactor, a dihydrolipoamide acyltransferase (E2) with a lipoate cofactor, and a dihydrolipoamide dehydrogenase (E3) with a flavin cofactor. E1 binds the 2-oxobutanoate to the lipoate on E2, which then transfers the propionyl group to coenzyme A, producing propionyl-CoA and reducing the lipoate. E3 then transfers protons to NAD in order to restore the lipoate. Propionyl-CoA carboxylase transforms the propionyl-CoA to S-methylmalonyl-CoA, which is then converted to R-methylmalonyl-CoA via methylmalonyl-CoA epimerase. In the final step, methylmalonyl-CoA mutase acts on the R-methylmalonyl-CoA to produce succinyl-CoA.
|
|
||
SMP0000455 |
Homocysteine DegradationHomocysteine is an amino acid and homologue of cysteine that appears in the body as a result of the degradation of methionine. In mammals, homocysteine is used to biosynthesize cysteine via the following pathway. First the enzyme cystathionine beta-synthetase irreversibly condenses homocysteine with L-serine, forming L-cystathionine. The L-cystathionine is then cleaved by cystathionine gamma-lyase, producing 2-oxobutanoate, L-cysteine, and ammonia. The 2-oxobutanoate is further broken down via the 2-oxobutanoate degradation pathway, producing citric acid cycle intermediates, while the L-cysteine goes to the cysteine metabolism pathway. The homocysteine degradation pathway composes a part of the larger methionine metabolism pathway.
|
|||
SMP0000450 |
Phytanic Acid Peroxisomal Oxidation Phytanic acid, a branched chain fatty acid, is an important component of fatty acid intake, occuring in meat, fish and dairy products. Due to its methylation, it cannot be a substrate for acyl-CoA dehydrogenase and cannot enter the mitochondrial beta oxidation pathway. Phytanic acid is instead activated to its CoA ester form by a CoA synthetase to phytanoyl-CoA, where it can begin the first cycle of alpha oxidation. Phytanoyl-CoA is a substrate for a specific alpha-hydroxylase (Phytanoyl-CoA hydroxylase), which adds a hydroxyl group to the α-carbon of phytanic acid, creating the 19-carbon homologue, pristanic acid. Pristanic acid then undergoes further metabolism through beta oxidation.
|
|
||
SMP0000006 |
Tyrosine MetabolismThe tyrosine metabolism pathway describes the many ways in which tyrosine is catabolized or transformed to generate a wide variety of biologically important molecules. In particular, tyrosine can be metabolized to produce hormones such as thyroxine and triiodothyronine or it can be metabolized to produce neurotransmitters such as L-DOPA, dopamine, adrenaline, or noradrenaline. Tyrosine can also serve as a precursor of the pigment melanin and for the formation of Coenzyme Q10. Additionally, tyrosine can be catabolized all the way down into fumarate and acetoacetate. This particular pathway for tyrosine degradation starts with an alpha-ketoglutarate-dependent transamination reaction of tyrosine, which is mediated through the enzyme known as tyrosine transaminase. This process generates p-hydroxyphenylpyruvate. This aromatic acid is then acted upon by p-hydroxylphenylpyruvate-dioxygenase which generates the compound known as homogentisic acid or homogentisate (2,5-dihydroxyphenyl-1-acetate). In order to split the aromatic ring of homogentisate, a unique dioxygenase enzyme known as homogentisic acid 1,2-dioxygenase is required. Through this enzyme, maleylacetoacetate is created from the homogentisic acid precursor. The accumulation of excess homogentisic acid and its oxide (named alkapton) in the urine of afflicted individuals can lead to a condition known as alkaptonuria. This genetic condition, also known as an inborn error of metabolism or IEM, occurs if there are mutations in the homogentisic acid 1,2-dioxygenase gene. After the breakdown of homogentisate is achieved, maleylacetoacetate is then attacked by the enzyme known as maleylacetoacetate-cis-trans-isomerase, which generates fumarylacetate. This isomerase catalyzes the rotation of the carboxyl group created from the hydroxyl group via oxidation. This cis-trans-isomerase uses glutathione as a coenzyme or cofactor. The resulting product, fumarylacetoacetate, is then split into acetoactate and fumarate via the enzyme known as fumarylacetoacetate-hydrolase through the addition of a water molecule. Through this set of reactions fumarate and acetoacetate (3-ketobutyroate) are liberated. Acetoacetate is a ketone body, which is activated with succinyl-CoA, and thereafter it can be converted into acetyl-CoA, which in turn can be oxidized by the citric acid cycle (also known as the TCA cycle) or used for fatty acid synthesis. Other aspects of tyrosine metabolism include the generation of catecholamines. In this process, the enzyme known as tyrosine hydroxylase (or AAAH or TYH) catalyzes the conversion of tyrosine to L-DOPA. The L-DOPA can then be converted via the enzyme DOPA decarboxylase (DDC) to dopamine. Dopamine can then be converted to 3-methoxytyramine via the action of catechol-O-methyltransferase (COMT). Dopamine can also be converted to norepinephrine (noradrenaline) through the action of the enzyme known as dopamine beta hydroxylase (DBH). Norepinephrine can then be converted to epinephrine (adrenaline) through the action of phenyethanolamine N-methyltransferase (PNMT). Catecholamines such as L-DOPA, dopamine and methoxytyramine are produced mainly by the chromaffin cells of the adrenal medulla and by neuronal cells found in the brain. For example, dopamine, which acts as a neurotransmitter, is mostly produced in neuronal cell bodies in the ventral tegmental area and the substantia nigra while epinephrine is produced in neurons in the human brain that express PMNT. Catecholamines typically have a half-life of a few minutes in the blood. They are typically degraded via catechol-O-methyltransferases (COMT) or by deamination via monoamine oxidases (MAO). Another important aspect of tyrosine metabolism includes the production of melanin. Melanin is produced through a mechanism known as melanogenesis, a process that involves the oxidation of tyrosine followed by the polymerization of these oxidation by-products. Melanin pigments are produced in a specialized group of cells known as melanocytes. There are three types of melanin: pheomelanin, eumelanin, and neuromelanin of which eumelanin is the most common. Melanogenesis, especially in the skin, is initiated through the exposure to UV light. Melanin is the primary pigment that determines skin color. Melanin is also found in hair and the pigmented tissue underlying the iris. The first step in the synthesis for both eumelanins and pheomelanins is the conversion of tyrosine to dopaquinone by the enzyme known as tyrosinase. The resulting dopaquinone can combine with cysteine to produce cysteinyldopa, which then polymerizes to form pheomelanin. Dopaquinone can also form lecuodopachrome, which then can be converted to dopachrome (a cyclization product) and this eventually becomes eumelanin. Tyrosine plays a critical role in the synthesis of thyroid hormones. Thyroid hormones are produced and released by the thyroid gland and include triiodothyronine (T3) and thyroxine (T4). These two hormones are responsible for regulating metabolism. Thyroxine was discovered and isolated by Edward Calvin Kendall in 1915. Thyroid hormones are produced by the follicular cells of the thyroid gland through the action of thyroperoxidase, which iodinates reactive tyrosine residues on thyroglobulin. Proteolysis of the thyroglobulin in cellular lysosomes releases the small molecule thyroid hormones. In mammals, tyrosine can be formed from dietary phenylalanine by the enzyme phenylalanine hydroxylase, found in large amounts in the liver. Phenylalanine is considered an essential amino acid, while tyrosine (which can be endogenously synthesized) is not. In plants and most microbes, tyrosine is produced via prephenate, an intermediate that is produced as part of the shikimate pathway.
|
|
|
|
SMP0000043 |
Galactose MetabolismThis pathway depicts the conversion of galactose into glucose, lactose, and other sugar intermediates that may be used for a range of metabolic process.
Dietary sources of galactose are numerous, but some of the primary sources in the human diet can be found in milk and milk derivative products.
This is because during digestion milk sugars and lactose are hydrolyzed into their molecular constituents (e.g. base monosaccharides). In milk, such monosaccharides include glucose and galactose.
The metabolism of the sugar Galactose is occurs almost entirely in the liver, and its metabolism is the consequence of three steps or reactions. First, the phosphorylation of galactose is induced by a special enzyme with the predictable name, galactokinase, and produces galactose 1-phosphate. Second, this biproduct and a second molecule, UDP-glucose, undergo a reaction which leads to the formation of UDP-galactose and glucose 1-phosphate. Thus, this reaction produces 1 molecule of glucose 1-phosphate per molecule of galactose.
This is mediated by the enzyme galactose-1-phosphate uridylyltransferase (GALT). The resulting UDP-galactose undergoes epimerization to form UDP-glucose via the enzyme UDP-galactose-4 epimerase (GALE). The UDP-glucose can be used in glucuronidation reactions and other pentose interconversions. In a reaction shared with other pathways, glucose 1-phosphate can be converted into glucose 6-phosphate. There are other pathways associated with galactose metabolism. For instance, galactose can be converted into UDP-glucose by the sequential activities of GALK, UDP-glucose pyrophosphorylase 2 (UGP2), and GALE. Galactose can also be reduced to galactitol by NADPH-dependent aldose reductase. Also shown in this pathway is
the conversion of glucose to galactose vis a vis a different process to the ones described earlier. This pathway, called hexoneogenesis, allows mammary glands to produce galactose. It should be noted however, that despite the existence of this pathway of galactose production, the vast majority of galactose in breast milk is actually the result of direct uptake up from the blood, whereas only a small fraction, ~35%, is the result of this de novo process hexoneogenesis.
Also depicted in this pathway are the conversions of other dietary di and tri-saccharides (raffinose, manninotriose, melibiose, stachyose) into galactose, glucose and fructose as well as and dietary sugar alcohols (melibitol, galactinol, galactosylglycerol) into sorbitol, myo-inositol, and glycerol.
|
|
|
|
SMP0000482 |
Mitochondrial Beta-Oxidation of Long Chain Saturated Fatty Acids Fatty acids and their CoA byproducts can be found in many places in the body, playing major roles in many basic functions of the body. These include signalling roles, energy creation roles and enzyme regulation. Beta-oxidation is a process that occurs in the peroxisomes and in the mitochondria, although this pathway is focused on the mitochondrial piece of that process. Depending on the length of the fatty acid, beta-oxidation will either begin in the peroxisomes or the mitochondria. Very long chain fatty acids, fatty acids that consist of more than 22 carbons, can be reduced in the peroxisome where they become octanyl-CoA before moving to the mitochondria for the rest of the oxidation process. Stearoylcarnitine is transported by a mitochondrial carnitine/acylcarnitine carrier protein into the mitochondrial matrix, where it is converted to stearoyl-CoA through the enzyme carnitine o-palmitoyltransferase 2. Stearoyl-CoA then is catalyzed into (2E)-octadecenoyl-CoA by the enzyme long-chain specific acyl-CoA dehydrogenase. Then, enoyl-CoA hydratase converts (2E)-octadecenoyl-CoA into (s)-hydroxyoctadecanoyl-CoA. The pathway continues as hydroxyacyl-coenzyme A dehydrogenase cleaves (s)-hydroxyoctadecanoyl-CoA into 3-oxooctadecanoyl-CoA. 3-oxooctadecanoyl-CoA then uses 3-ketoacyl-CoA thiolase to create acetyl-CoA (necessary for the citric acid cycle) and uses trifunctional enzyme subunits alpha and beta to create palmityl-CoA. This palmityl-CoA is then converted by long-chain specific acyl-CoA dehydrogenase to (2E)-hexadecenoyl-CoA. Enoyl-CoA then converts (2E)-hexadecenoyl-CoA to 3-hydroxyhexadecanoyl-CoA, which is then turned into 3-oxohexadecanoyl-CoA by the enzyme hydroxyacyl-coenzyme A dehydrogenase. 3-ketoacyl-CoA thiolase then creates acetyl-CoA with the help of trifunctional enzyme subunits alpha and beta, which also produce tetradecanoyl-CoA from 3-oxohexadecanoyl-CoA. Long-chain specific acyl-CoA dehydrogenase then converts tetradecanoyl-CoA to (2E)-tetradecenoyl-CoA. (2E)-tetradecenoyl-CoA is then converted by the enzyme enoyl-CoA hydratase into 3-hydroxytetradecanoyl-CoA, which then creates 3-oxotetradecanoyl-CoA through the enzyme hydroxyacyl-coenzyme A dehydrogenase. Finally, the 3 enzymes 3-ketoacyl-coA thiolase, trifunctional enzyme subunit alpha and trifunctional enzyme subunit beta convert 3-oxotetradecanoyl-CoA into acetyl-CoA and lauroyl-CoA which can then be beta-oxidized as medium chain saturated fatty acids.
|
|
|
|
SMP0000466 |
Transfer of Acetyl Groups into MitochondriaAcetyl-CoA is an important molecule, which is precursor to HMG CoA, which is a vital component in cholesterol and ketone synthesis. Acetyl CoA participates in the biosynthesis of fatty acids and sterols, in the oxidation of fatty acids and in the metabolism of many amino acids. It also acts as a biological acetylating agent. Acetyl-CoA is made in the mitochondria by metabolizing fatty acids, and the oxidation of pyruvate of acetyl-CoA. When the body has an excess of ATP, the energy in acetyl-Coa can be stored in the form of fatty acids. Acetyl-CoA must cross the mitochondrial membrane to the cytosol, where fatty acid synthesis takes place. Acetyl-CoA is combined with oxalacetic acid by the enzyme citrate synthase, creating citric acid. Citric acid is then transported out of the mitochondria, to the cytosol, where the enzyme citrate lyase converts citric acid back into acetyl-CoA and oxalacetic acid. Malate dehydrogenase reduces oxalacetic acid to malate, which then is either transported back into the mitochondria by the malate-alpha ketoglutarate transporter or oxidized to pyruvate by malic enzyme. Pyruvate can then be transported back into the mitochondria and undergo decarboxylation into oxalacetic acid. Malate can also be used to create NADH by the conversion of malate to oxalacetic acid by malate dehydrogenase.
|
|
||
SMP0000459 |
Pyruvaldehyde DegradationThis Pyruvaldehyde degradation pathway (Methylglyoxal degradation;2-oxopropanal degradation), also known as the glyoxalase system, is probably the most common pathway for the degradation of pyruvaldehyde (methylglyoxal), a potentially toxic metabolite due to its interaction with nucleic acids and other proteins. Pyruvaldehyde is formed in low concentrations by glycolysis, fatty acid metabolism and protein metabolism. Pyruvaldehyde is catalyzed by the glyoxylase system, composed of the enzymes lactoylglutathione lyase (glyoxalase I) and glyoxylase II. Glyoxalase I catalyes the isomerization of the spontaneously formed hemithioacetal adduct between glutathione and pyruvaldehyde into S-lactoylglutathione. S-lactoylglutathione is then catalyzed by glyoxalase II into D-lactic acid and glutathione. D-lactic acid is then catalyzed by an unknown quinol in the membrane to pyruvic acid, which then enters pyruvate metabolism.
|
|||
SMP0000589 |
Gastric Acid ProductionGastric acid plays a key role in the digestion of proteins by activating digestive enzymes to break down long chains of amino acids. In addition, it aids in the absorption of certain vitamins and minerals and also acts as one of the body's first line of defence by killing ingested micro-organisms. This digestive fluid is formed in the stomach (specifically by the parietal cells) and is mainly composed of hydrochloric acid (HCl). However, it is also constituted of potassium chloride (KCl) and sodium chloride (NaCl). The main stimulants of acid secretion are histamine, gastrin, and acetylcholine which all, after binding to their respective receptors on the parietal cell membrane, trigger a G-protein signalling cascade that causes the activation of the H+/K+ ATPase proton pump. As a result, hydrogen ions are able to be pumped out of the parietal cell and into the lumen of the stomach. The hydrogen ions are available inside the parietal cell after water and carbon dioxide combine to form carbonic acid(the reaction is catalyzed by the carbonic anhydrase enzyme) which dissociates into a bicarbonate ion and a hydrogen ion. Moreover, the chloride and potassium ions are transported into the stomach lumen through their own channels so that hydrogen ions and/or potassium ions can form an ionic bond with chloride ions to form HCl and/or KCl, which are both constituents of stomach acid. In addition, the peptide hormone somatostatin is the main inhibitor to gastric acid secretion. Not only does it inhibit the G-protein signalling cascade that leads to proton pump activation, but it also directly acts on the enterochromaffin-like cells and G cells to inhibit histamine and gastrin release, respectively.
|
Physiological
|
|
|
SMP0000715 |
Methylhistidine MetabolismMethylhistidine is a modified amino acid that is produced in myocytes during the methylation of actin and myosin. It is also formed from the methylation of L-histidine, which takes the methyl group from S-adenosylmethionine and forms S-adenosylhomocysteine as a byproduct.
After its formation in the myocytes, methylhistidine enters the blood stream and travels to the kidneys, where it is excreted in the urine.
Methylhistidine is present in the blood and urine in higher concentrations after skeletal muscle protein breakdown, which can occur due to disease or injury. Because of this, it can be used to judge how much muscle breakdown is occurring. Methylhistidine levels are also affected by diet, and may differ between vegetarian diets and those containing meats.
|
|
Showing 101 -
110 of 142613 pathways